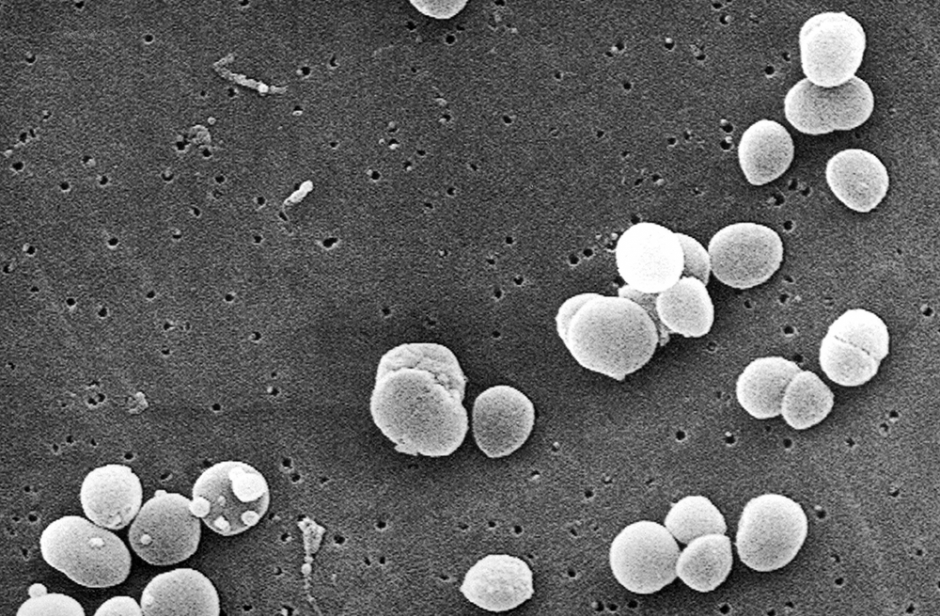
A mechanobiological discovery made by using an atomic force microscope creates prospects in the fight against nosocomial diseases caused by staphylococci.
Sometimes referred to as a ‘hospital killer’, golden staphylococcus (Staphylococcus aureus) is, along with another well-known bacterium, Escherichia coli, responsible for the majority of nosocomial diseases: diseases that are contracted in hospital. Staphylococci, which are often found in dirty water, soils, etc., and can also be responsible for food poisoning, are regulars on our skin and external mucosa: according to the Institut Pasteur, 30 to 50% of humans are healthy carriers of staphylococci, meaning they’re carriers of the bacteria without developing disease. The bacteria are therefore considered members of our natural bacterial flora.
Then when do they cause infections? Apart from contaminated food ingestion, which doesn’t concern us here, contaminations can occur during injuries, when the skin is broken, and surgery. The resulting pathologies are, however, most often controlled by antibiotic treatment. But not always. Firstly because many staphylococcus strains have become resistant to this type of treatment, but secondly because staphylococci have the unfortunate tendency to form biofilms, which for years have been the target of Professor Yves Dufrêne, an FNRS research director at UCL’s Louvain Institute of Biomolecular Science and Technology (LIBST) as well as a Welbio researcher.
‘Biofilms are communities of bacteria that can contain several billion of them’, Prof. Dufrêne explains. ‘They adhere to the surfaces of human tissues but also the surfaces of medical devices such as catheters, probes or prostheses before secreting a coating, multiplying, joining together and forming a very sticky layer that’s very difficult to eradicate’.
It’s indeed curious that while individual bacteria are susceptible to antibiotics, as biofilm, they resist. Biofilms are considered responsible for 60 to 80% of nosocomial infections. The fight against them has become a major medical issue. ‘The challenge of our research,’ Prof. Dufrêne says, ‘is to block the adhesion of bacteria rather than using antibiotics. They don’t adhere by chance, they have developed on the surface of key proteins, called adhesins or adhesion proteins, that have a very specific ‘key-lock’ interaction. Disabling these makes the bacteria non-adherent and that’s what we’re trying to achieve.’
Mechanobiology
In 2015, Prof. Dufrêne’s team discovered that a protein located on the surface of staphylococcus, SasG, plays a role in the adhesion of bacteria to each other: in the presence of zinc, SasG behaves like a Velcro band which attaches to the Velcro of nearby bacteria. Last year, his team took a big step forward, identifying a molecule capable of inactivating a major adhesion protein that allows staphylococci to form biofilms. It’s a peptide derived from the neuronal molecule β-neurexin. These two major discoveries were made possible thanks to the development of a new field of biology: mechanobiology.
The field took off around 20 years ago and involves studying how cells react to mechanical forces. It was born out of a question: How do we understand the mode of action of cells in their biochemical environment if we neglect their ability to exert mechanical forces and react to them? This was essential, because cells use these tensions and pressures to adapt to their environment, for example by migrating or otherwise adhering to a material. In fact, the interactions of cells with each other or with their environment are influenced by the mechanical stresses exerted on them. Thus, for example, epithelial cells, such as those that make up skin or intestinal walls, have been observed communicating via mechanical forces to coordinate their movements. Another example: researchers have found that stretching cells increases the activity of two intracellular proteins (YAP and TAZ), causing uncontrollable cell replication, as if they were becoming ‘cancerous’. Still another: cells that have space around them continue to divide, while those that are confined do so much more slowly. It’s understandable that this area of biology increasingly targets areas as varied as oncology, embryo development, scarring, and, more recently, microbiology, and, more particularly, the formation of bacterial biofilms.
Atomic force microscope
These advances have been possible thanks to technological developments in live-cell nanoscopy. ‘In our work,’ Prof. Dufrêne explains, ‘as in the work of many others in mechanobiology, we must observe the behaviour of proteins, which are much smaller than cells. On the order of one nanometre, one-millionth of a millimetre, which is not observable by optical microscopes.’
In optical microscopy, magnification is limited by the wavelength of light. Electron microscopy, first introduced in 1931, allows much higher magnifications, using electrons rather than photons. More recently, new microscopies ‘with local probes’ have appeared, allowing for the first time to ‘see’ and ‘feel’ atoms and molecules. The principle is radically different from that of other microscopes because, instead of using incident radiation (photons, electrons), a microscopic probe is moved at a very short distance from the surface to be analysed. Thus, in biology, the atomic force microscope (AFM) allows for the observation of life at the nanoscale as well as directly in a liquid medium, which the electron microscope doesn’t allow. Invented in 1986 by the physicists Heinrich Rohrer, Gerd Binning and Calvin Quate, the AFM performs 3D mapping and describes the physical properties of a material at the nanoscale. To achieve this, it detects atomic forces between the surface of the sample and a probe, or tip, that moves at a very short distance above it. The tip, which must be as minute as possible, is at the end of a flexible cantilever that records any disturbances and – especially interesting to us here – measures observed forces.
Stress increases strength tenfold
‘If the bacteria join each other or walls’, Prof. Dufrêne said, ‘it’s because proteins on their surface develop adhesion forces. Our goal was to measure these forces through the AFM to better understand how these proteins work. It is exceptional to achieve this with live bacteria.’ With what results? At rest, the forces observed are obviously very low (on the order of the piconewton (10-12N)), but this value is multiplied by several orders of magnitude when the protein is ‘stressed’, for example when it’s stretched. Explains Prof. Dufrêne, ‘We then reach values equivalent to a covalent bond. Measuring this in living cells is quite exceptional and unexpected.’ In other words, the researchers have observed a major phenomenon: the adhesiveness of adhesion proteins is strongly enhanced when they’re subjected to mechanical stress. Yet this stress is exactly what characterises the daily lives of our staphylococci: whether in the bloodstream, on catheters, or on skin (when we scratch ourselves, for example) or even when they multiply into biofilms, they’re subject to turbulence and shocks.
In their latest study published in the journal PNAS(1) and carried out with colleagues from Trinity College Dublin, the researchers were interested in a particular adhesion protein, ClfA, which has the particularity of functioning in ‘key-lock’ interaction with fibrinogen, a blood plasma protein widespread on medical devices. In the event of mechanical tension or stress, this key-lock mechanism that binds the two types of proteins is considerably strengthened and the staphylococcus adheres more than ever to the biomaterial. The study was funded by Welbio and the European Research Council.
Henri Dupuis
(1)Staphylococcus aureus clumping factor A is a force-sensitive molecular switch that activates bacterial adhesion, Ph. Herman-Bausier et al., PNAS, May 7, 2018. https://doi.org/10.1073/pnas.1718104115
AFM operation
This microscope does not use incident rays but a probe consisting of a lever ending in the finest point possible, ideally a single atom. If this atom-tip is close to the atoms of the surface of the sample, a repulsive force will be generated which increases rapidly with the inverse of the distance because the electronic clouds of the ions will overlap. If, however, tip and sample are more distant (but ‘aware’ of each other’s presence), an attractive force (called Van der Waals) will bring them closer together. By moving the tip over the sample (‘scanning’ the sample), one thus obtains a succession of attractions and repulsions which is clearly recorded by the lever and generates a 3D map of the surface.
A glance at Yves Dufrêne's bio
1991 Graduated from Faculty of Bioscience Engineering, UCL
1996 Doctoral thesis, UCL
1996-97 Post-doctorate at Naval Research Laboratory (US)
Since 2000 FNRS Researcher (Senior Research Associate), Institute of Life Sciences, and Professor in the Faculty of Bioscience Engineering, UCL
2009 Winner, Prix Pacault, Société française de Chimie (‘French Society of Chemistry’)
2012 Winner, Quadrennial Life Sciences Award, European Microscopy Society
2013 Winner, Prix Léo Errera, Royal Academy of Belgium
2015 Associate Editor, Nanoscale
2016 Winner, European Research Council Advanced Grant for research project NanoStaph